What is a lithium-ion battery and how does it work?
The lithium-ion (Li-ion) battery is the predominant commercial form of rechargeable battery, widely used in portable electronics and electrified transportation. The rechargeable battery was invented in 1859 with a lead-acid chemistry that is still used in car batteries that start internal combustion engines, while the research underpinning the Li-ion battery was published in the 1970s and the first commercial Li-ion cell was made available in 1991. In 2019, John B. Goodenough, M. Stanley Whittingham, and Akira Yoshino received the Nobel Prize in Chemistry for their contributions to the development of the modern Li-ion battery.
During a discharge cycle, lithium atoms in the anode are ionized and separated from their electrons. The lithium ions move from the anode and pass through the electrolyte until they reach the cathode, where they recombine with their electrons and electrically neutralize. The lithium ions are small enough to be able to move through a micro-permeable separator between the anode and cathode. In part because of lithium’s small atomic weight and radius (third only to hydrogen and helium), Li-ion batteries are capable of having a very high voltage and charge storage per unit mass and unit volume.
Li-ion batteries can use a number of different materials as electrodes. The most common combination is that of lithium cobalt oxide (cathode) and graphite (anode), which is used in commercial portable electronic devices such as cellphones and laptops. Other common cathode materials include lithium manganese oxide (used in hybrid electric and electric automobiles) and lithium iron phosphate. Li-ion batteries typically use ether (a class of organic compounds) as an electrolyte.
Lithium ions are stored within graphite anodes through a mechanism known as intercalation, in which the ions are physically inserted between the 2D layers of graphene that make up bulk graphite. The size of the ions relative to the layered carbon lattice means that graphite anodes are not physically warped by charging or discharging, and the strength of the carbon-carbon bonds relative to the weak interactions between the Li ions and the electrical charge of the anode make the insertion reaction highly reversible.
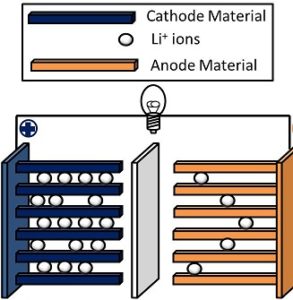
Battery Applications
Mathematical Models for Battery Efficiency
What are some advantages of Li-ion batteries?
Compared to other high-quality rechargeable battery technologies (nickel-cadmium, nickel-metal-hydride, or lead-acid), Li-ion batteries have a number of advantages. They have some of the highest energy densities of any commercial battery technology, as high as 330 watt-hours per kilogram (Wh/kg), compared to roughly 75 Wh/kg for lead-acid batteries. In addition, Li-ion cells can deliver up to 3.6 volts, 1.5–3 times the voltage of alternatives, which makes them suitable for high-power applications like transportation. Li-ion batteries are comparatively low maintenance, and do not require scheduled cycling to maintain their battery life. Li-ion batteries have no memory effect, a detrimental process where repeated partial discharge/charge cycles can cause a battery to ‘remember’ a lower capacity. Li-ion batteries also have a low self-discharge rate of around 1.5–2% per month, and do not contain toxic lead or cadmium.
High energy densities and long lifespans have made Li-ion batteries the market leader in portable electronic devices and electrified transportation, including electric vehicles (EVs) like the Nissan Leaf and the Tesla Model S as well as the hybrid-electric Boeing 787. In terms of decarbonizing our economy’s energy use, Li-ion technology has its greatest potential in EVs and electrified aviation.
What are some disadvantages of Li-ion batteries?
Not only are lithium-ion batteries widely used for consumer electronics and electric vehicles, but they also account for over 80% of the more than 190 gigawatt-hours (GWh) of battery energy storage deployed globally through 2023. However, energy storage for a 100% renewable grid brings in many new challenges that cannot be met by existing battery technologies alone.
First, more than 10 terawatt-hours (TWh) of storage capacity is needed, and multiplying today’s battery deployments by a factor of 100 would cause great stress to supply chains of rare materials like lithium, nickel and cobalt. Second, large-scale, long-duration energy storage requires extremely low costs — significantly less than $100/kWh, or more than twice as cheap today’s state-of-the-art battery technologies — and more than 20 years of reliable service life. Furthermore, scaling up conventional battery energy storage systems from kWh to MWh or GWh presents a serious challenge for robust electric and thermal management.
For the U.S to store 8 hours of electricity, it would need to deploy terawatt-hours of batteries, which would cost trillions of dollars at today’s prices, while 6 weeks of seasonal heating would require petawatt-hours (thousands of TWh) of storage. Therefore, a 100% clean energy future requires not only the development of low-cost battery technologies using environmentally friendly, earth-abundant materials, but also new storage strategies using a combination of electrochemical, chemical, thermal and mechanical mechanisms.
CEI Research Highlights
A major focus of CEI energy storage research is the development of novel materials to improve battery performance. Some CEI researchers develop substitutes for the components of a conventional Li-ion battery, such as silicon-based anodes instead of graphite. Others work to improve upon well-developed battery components by building in micro- and nano-scale architectures that can improve the speed and efficiency of charge cycles, with physical features that are smaller than the width of a human hair. CEI researchers are also exploring alternative chemistries to Li-ion that might be suitable for a specific application.
For example, chemical engineering (ChemE) professor Vincent Holmberg and his research group are developing and investigating alloying materials for Li-ion batteries. Materials like silicon, germanium, and antimony react with Li ions to form alloys, which results in greater capacities than graphite anodes that rely on intercalating Li ions between layers of graphene. However, alloying materials experience greater changes in physical volume that can deform the electrode and lead to performance losses or failure. But by introducing a nanostructure into the alloying material, the Holmberg group can reduce the stress and strain on the electrode from the charge and discharge reactions. The physical morphologies of the electrodes can affect the battery’s ability to hold and transfer charge, as can any chemical interactions between the lithium ions and the surface of the electrodes.
Developing a deeper understanding of reversible “conversion” charge-discharge reactions is key to deploying new battery chemistries with higher theoretical energy densities, such as lithium-sulfur. With sulfur’s abundance and relatively low atomic weight, Li-S batteries could be cheaper and lighter than Li-ion batteries with graphite anodes, but achieving this high energy density simultaneously with long cycle life remains a grand challenge for energy storage scientists and engineers. Lithium-based devices often fail due to the formation of “dendrites” of lithium metal growing on the anodes like tree roots through a sidewalk.
Materials science & engineering professor Jun Liu investigates the degradation mechanisms of Li metal with Li-nickel manganese cobalt (NMC) cathodes in pouch cells, and has presented fundamental linkages among Li thickness, electrolyte depletion, and the structural evolution of solid–electrolyte interphase layers. Meanwhile, CEI director and ChemE professor Dan Schwartz and his group are working on computational models of Li-S systems that can be corroborated by experimental results. Liu is the director of the Battery500 Consortium — led by the Pacific Northwest National Laboratory (PNNL) and including Schwartz on the executive committee — which aims to develop next-generation EV batteries with energy densities approaching 500 watt-hours per kilogram, double the industry standard.
With technological progress in mobile electronics driving demand for denser batteries, engineers are also employing three-dimensional (3D) electrode architectures and additive manufacturing methods to rapidly fabricate battery prototypes with improved performance. Research led by mechanical engineering (ME) professor Corie Cobb in her Integrated Fabrication Lab focuses on how 3D electrode architectures can improve many aspects of battery performance. Furthermore, with the state-of-the-art prototyping and testing capabilities at the Washington Clean Energy Testbeds, ME and materials science & engineering (MSE) professor J. Devin MacKenzie’s group and the Holmberg group are collaborating to structurally engineer antimony alloying electrodes. Special inkjet printers allow these engineers to build 3D electrode architectures with droplets just microns across, while one of the only open-access, high-throughput roll-to-roll electronics printers in the world enables rapid iteration at commercial scales. The Testbeds, at which MacKenzie is technical director, also house top-of-the-line microscopes and battery testing equipment to validate new electrode designs.
CEI researchers are also creating physical, mathematical and computational models to evaluate how batteries operate and fail. These models can help optimize battery performance and charge/discharge cycles and predict dangerous battery failures. The Schwartz group is advancing diagnostics for Li-ion batteries to obtain data on day-to-day operations and battery health, a dynamic alternative to a physical “autopsy” at the end of the device’s use. Along with physics-based models of battery systems, these diagnostic tools can detect signs of degradation in real time, allowing users to modify their operations to extend battery lifespans. Furthermore, researchers in the Schwartz group use these models to project second lives for batteries that have degraded beyond EV performance standards, such as in solar-powered microgrids.
With the UW “Hyak” supercomputer, researchers can simulate molecules and their kinetic and thermodynamic interactions to understand electrochemistry from a perspective that is not afforded to experimental techniques.
CEI researchers also use direct imaging techniques like x-ray spectroscopy to understand the inner workings of batteries. Professor Jerry Seidler’s lab has developed a method to perform X-ray absorption near edge structure (XANES) spectroscopy on the benchtop. The technique provides relatively detailed measurements of certain characteristics of a battery’s internal state, without having to open it and thus disrupt the system. Previously, XANES could only be accomplished with an extremely high radiative flux, from instruments such as a synchrotron. These are extremely large and expensive facilities, costing up to $1 billion and often only available to the public via federal labs with months-long waiting lists.. But as optoelectronic technologies have evolved, the Seidler lab spun out a company to prototype a $25,000 benchtop instrument that can mimic the measurements taken at a synchrotron. The EasyXAFS already enables scientists to obtain XANES measurements in hours, which can accelerate the innovation cycle for batteries and other energy-related materials and devices.
Meanwhile, chemistry professor Cody Schlenker and his group investigate the fundamental chemistry of interfaces within energy storage systems with the goal of gaining a deeper understanding of electrochemical processes. By coupling electrochemistry theory with spectroscopy, the lab can identify changes in vibrational frequencies and in the dynamics of ion transfer and link them to specific chemical phenomena at key interfaces between electrodes, separator membranes, and electrolytes.
Explore More
- Website on batteries and battery reuse created by Clean Energy Bridge to Research REU student Alek Lazarski